Generally, the worldwide use of space systems is broken down into three user communities: Commercial, Civil and Military. Each of these communities share common needs, interests and uses of space systems and services.
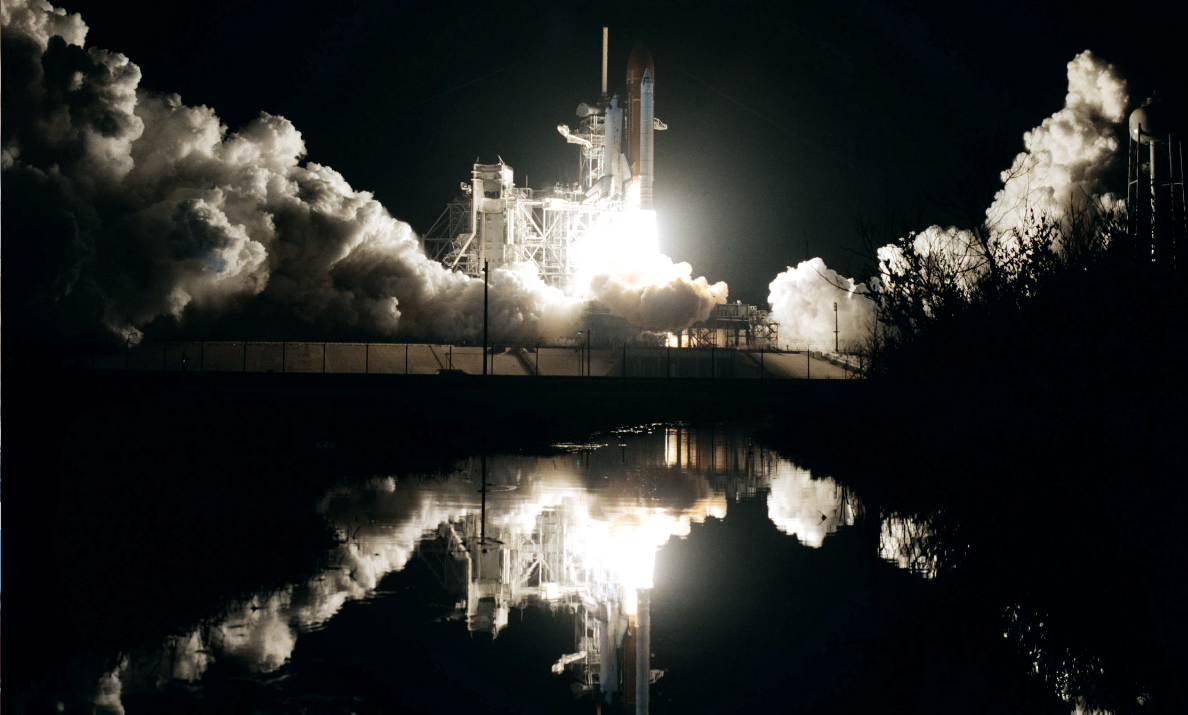
The commercial space user community is primarily interested in broadcast communications, point-to-point communications, position and navigation services, and imagery. The civil space community is composed of non-military and non-intelligence government agencies that use space.
In the U.S., the largest civil organizations engaged in space are NASA and NOAA. NASA is charged with exploring space, doing science missions focusing on the earth and our solar system, and developing technology for use in space. NOAA is responsible for weather monitoring and forecasting, which relies heavily on space-based assets.
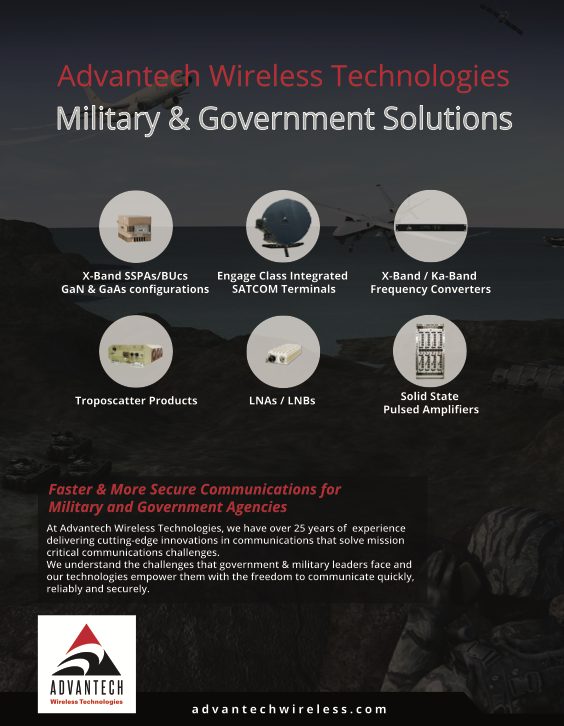
The military space community is composed of the armed forces and the intelligence agencies that use space as a medium from which to gather information or as an environment in which to execute operations. Intelligence users are interested in employing satellites to monitor activities in denied areas.
Military users are interested in satellites to help with navigation, weather forecasting, and worldwide communication, in addition to intelligence gathering to support specific military engagements.
For space architectures with multiple satellites, the launch segment plays an important role in mission risk reduction and constellation replenishment and maintenance strategies.
The ground segment often includes a choice of whether to use data downlink gateway systems in space (Tracking and Data Relay Satellite System) or on the earth (e.g., the Deep Space Network, or Air Force Satellite Control Network for continual execution of Telemetry, Tracking, and Commanding (TT&C) operations).
Also of consideration is where data processing will take place and how mission data will be stored and distributed. Assuming 50 pictures of 500 MB of high resolution imagery every day for 10 years, the space system architect has 91 terabytes of information to store (the equivalent of the U.S. Library of Congress’ entire contents). [1]
Space system design often starts with needs and a concept. Engineers perform trade studies by setting baselines and making minor changes to seek improvement in performance, cost, schedule, and risk. The culture of the industry that grew through an Apollo race to the moon and large defense contracts in the 1970s and 1980s was slow to adapt to better design systems.
Experience has shown that the design vector presents the least stable element in the trade space. Modular designs included as many variables as practical in a “constants” vector rather than “hardwiring” the values into code. These could be design variables were assumed to have weak impact, or variables that could conceivably change in the future but were not expected to impact the design. [2]
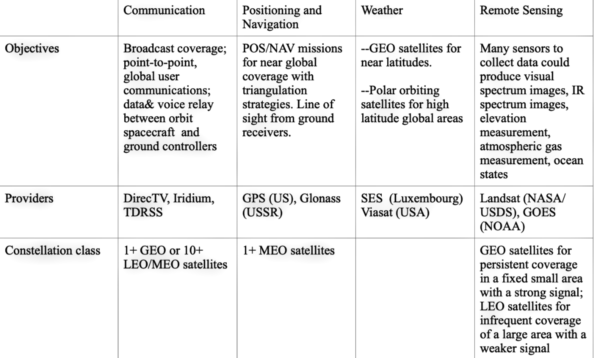
Orbit types and parameters are generally selected to provide the greatest benefit for the least cost, based on the purpose and capabilities of the satellite. The four most common orbit types used by the military are Geosynchronous Earth Orbit, Highly Elliptical Orbit, Medium Earth Orbit, and Low Earth Orbit (Figure 1). Generally speaking, space system architectures are classified by the mission performed.
The launch of constellations of small satellites providing high-band width data to terrestrial users was cost-prohibitive in the past, particularly with COMSATCOM. Satellites were optimized for a specific orbit based on function. This meant that a dedicated launch platform was required. Because launch costs were high and a functional satellite could only be built above a certain weight, a self-reinforcing incentive cycle developed. Miniaturization of satellite components and increased understanding of the space environment now allow functional platforms to be built that are significantly lighter than those of the past. [3]
The National Oceanic and Atmospheric Administration (NOAA) Satellite Observing System Architecture Study developed a satellite plan with broad trade-space regarding instruments, platforms, launch policy, and orbits likely available in a 2028 timeframe. Nearly 100 prototype constellations were developed based on different configurations of instruments and orbits. The forward-looking, quantitative, cost-benefit analysis was not intended to deliver a particular point solution with definitive satellite constellation, instrument vendor, or data service provider. Rather, it identified options to inform budget and program decisions. [4]
A new generation of cooperative spacecraft designed specifically for on-orbit servicing (OOS) missions could upgrade its hardware every few years—a need identified by the commercial, civil, and military satellite sectors. This would end the current paradigm of relying on monolithic-purposed satellites with decades-old hardware and technology, then having to launch replacements to modernize them. OOS refers to on-orbit activities conducted by a space vehicle that performs up-close inspection of, or results in intentional and beneficial changes to, another resident space object (RSO). These activities include non-contact support, orbit modification (relocation) and maintenance, refueling and commodities replenishment, upgrade, repair, assembly, and debris mitigation. [5]
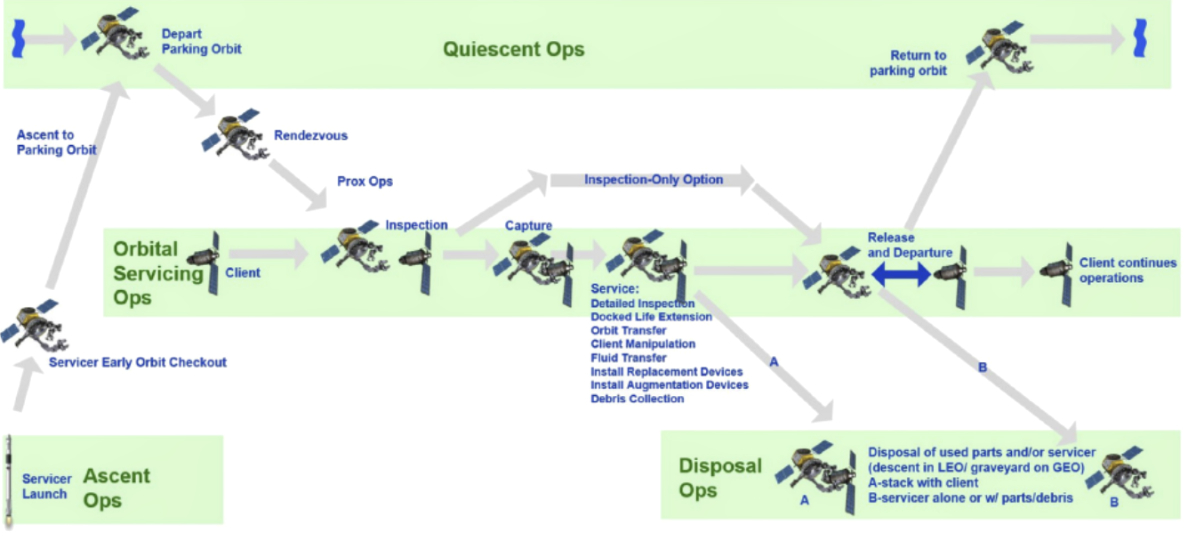
Click to enlarge.
Public-private partnerships have incentivized the OOS market with commercially driven services and technologies. Recent start-ups rooted in Silicon Valley business tactics have increasingly launched smaller and more affordable satellites for their constellations. With the sharp increase in spacecraft, the shift towards an always-connected, Internet-of-Things (IoT) mindset in space is paramount. In practice, satellites receive and direct data from millions of small, uncoordinated sensors from remote areas with low data rates. Flat antennas allow for IoT backhauling on any platform, including high throughput satellites or other fixed satellite services, which would allow for IoT to have a more significant presence.
With OOS support, Defense Advanced Research Projects Agency (DARPA) has pioneered the concept of using robots in space. Active Debris Removal (ADR) technology demonstrated the best way to capture the estimated 40,000 pieces of space debris orbiting Earth. In a public-partnership with Space Infrastructure Services (SIS), sophisticated satellite servicing was commercialized, including that of refueling.
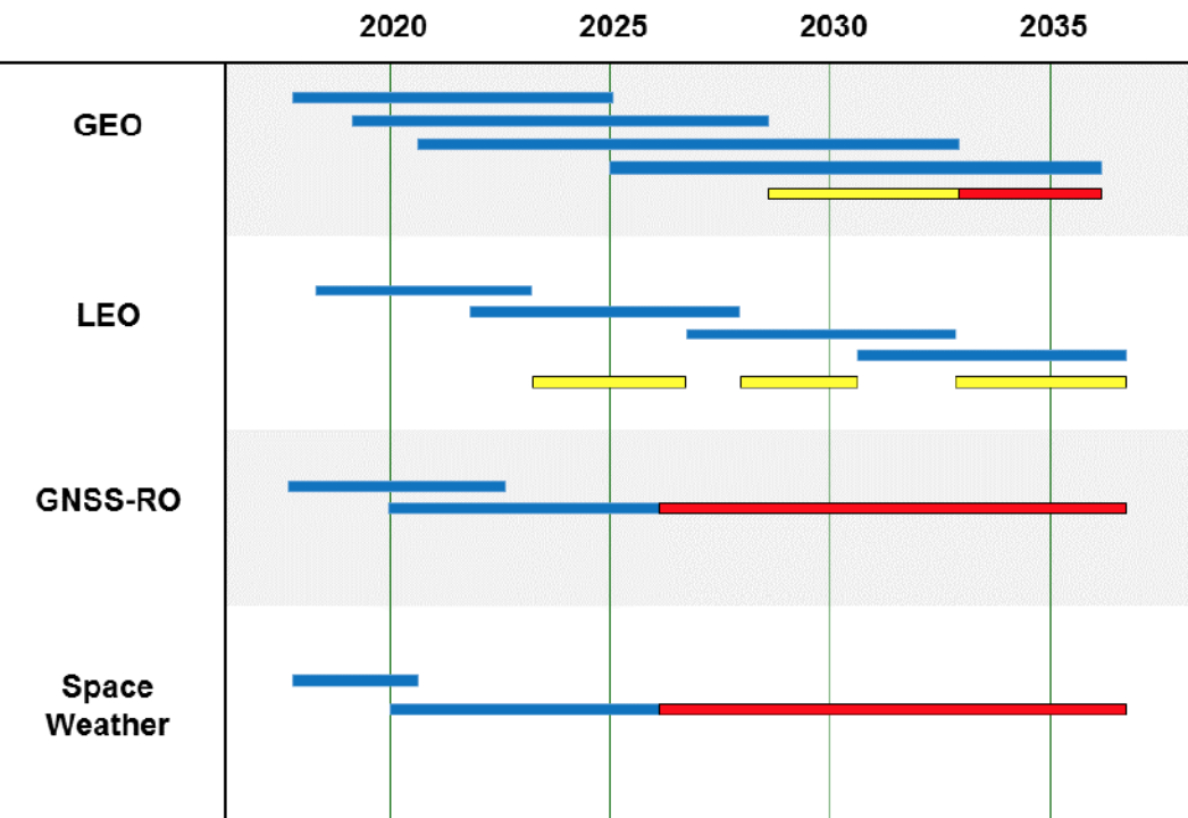
Moreover, SES Networks operated more than 50 geosynchronous satellites and 12 mid-Earth orbit satellites. Governments and commercial interests appear to be pivoting GEO-based capabilities to low earth orbit. In comparison to reduced station-keeping propellant, geostationary orbits afford mission planners a fixed sub-satellite point with the advantage for broader end-to-end mission. For example, mission designs indicate nearly continuous coverage. Still, GEO constellations fail to provide access to the planet’s polar regions, a driving requirement for some mission. [6]
NASA’s efforts to explore space have also been the driving factor for OOS development . Commercial resupply missions to ISS indicate a consistent need to perform rendezvous and proximity operations (RPO). If these missions are successful, the space industry will likely see a rapid growth of OOS capabilities resulting in reduced operating cost, including servicer use in satellite insurance contracts to mitigate or repair failures in lieu of replacing spacecraft.
The Consortium for Execution of Rendezvous and Servicing Operations (CONFERS) is an industry-led initiative with initial seed funding provided by the DARPA to leverage best practices from government and industry to research, develop, and publish non-binding, consensus- derived technical and operations, OOS- and RPO- standards [7]. Large government satellite programs, take 10-15 years from an initial satellite program concept to the first satellite reaching orbit depending on system complexity and other programmatic factors.
NOAA’s Global Navigation Satellite System Radio Occultation (GNSS-RO) and space weather capabilities require replenishment much earlier. Three satellites are simultaneously required on-Geo-orbit with two active (East and West) and a third as a spare (typically, centrally located over the U.S.).
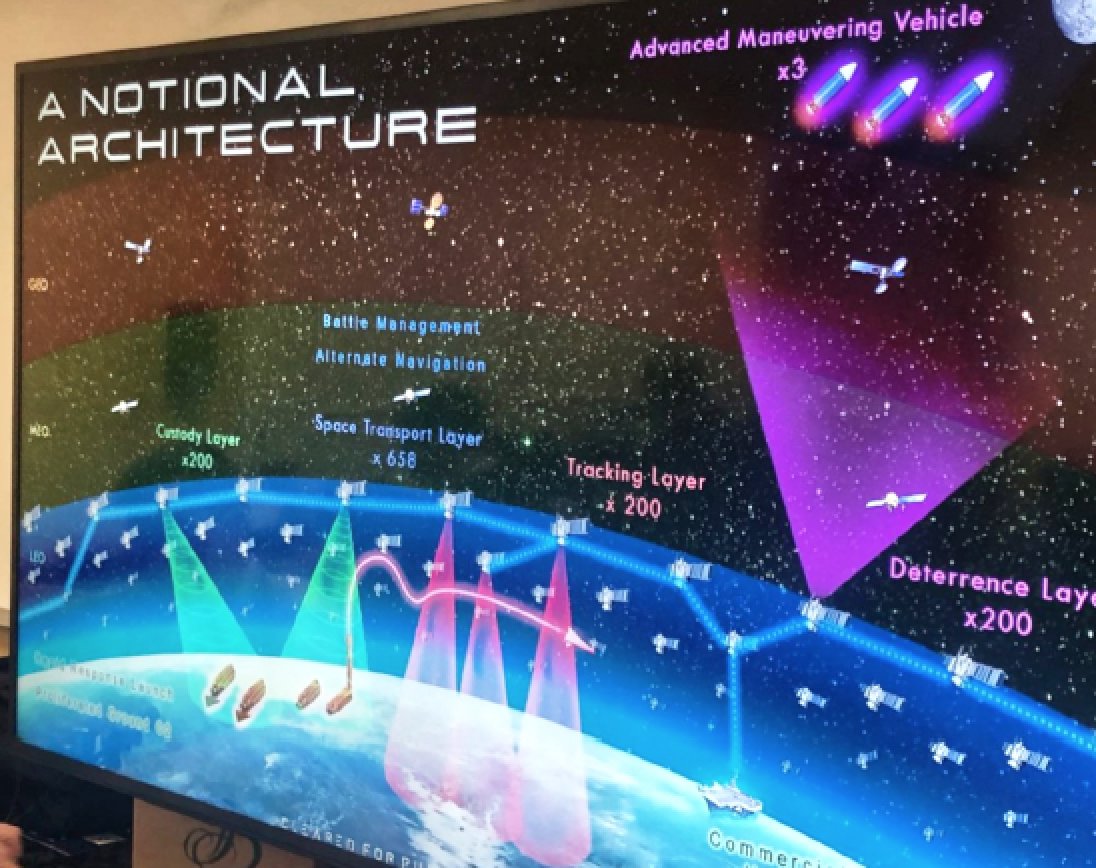
Space Defence Agancy "Notional" Space Architecture Reaches for the Moon.
Figure 3 above shows satellites GOES -15, GOES-17, and GOES-T, the anticipated flyout of GOES-17, replenishment capabilities availability for launch (yellow bar), and the loss of capability (early 2030s) after the GOES-T flyout (red bar). Blue lines indicate expected lifetime of launched or planned satellites or capabilities. Yellow bars indicate time periods when availability falls below the current policy. And, red bars indicate loss of capability. [8]
Smallsats are an emerging class of spacecraft that incorporates recent software and hardware improvements. When compared to traditional satellites, smallsats typically have shorter development cycles, smaller development teams, and consequently, lower cost, both for the development and for the launch of the satellites. CubeSats have the additional benefit of a standardized form-factor and containerization, enabling mass production and easier launch vehicle integration, which can further lower cost.
These lower-cost satellites’ display expendability, faster refresh, and simultaneous deployment in large numbers. Demand and subsequent investment in on-orbit servicing, assembly, and manufacturing (OSAM) is influenced by developments in both upstream and downstream fields (e.g., communications, Earth Observation (EO), space exploration, data analytics, private launch market, etc. [9]
GEO-Earth (Military) Notional Satellite Architecture Space is a naturally hazardous environment and is increasingly congested, contested, and competitive. Natural threats to satellites include solar activity, radiation belts, and natural orbital debris. Man-made threats can be both unintentional (e.g., satellite debris or electromagnetic interference) or intentional (e.g., jamming, lasing, cyberspace attacks, and anti-satellite weapons).
Nations are also developing, and in some cases demonstrating, disruptive and destructive space capabilities. Consequently, space situational awareness (SSA) functions as a defense strategy dependent on integrating space surveillance, collection, and processing; environmental monitoring; status of US and cooperative satellite systems; understanding of US and multinational space readiness; and analysis of the space domain. Since space assets provide combatant commanders (CCDRs) with near-worldwide coverage and access to otherwise denied areas, the advantages using space for operational purposes include freedom of action, overflight, and global perspective and responsiveness.
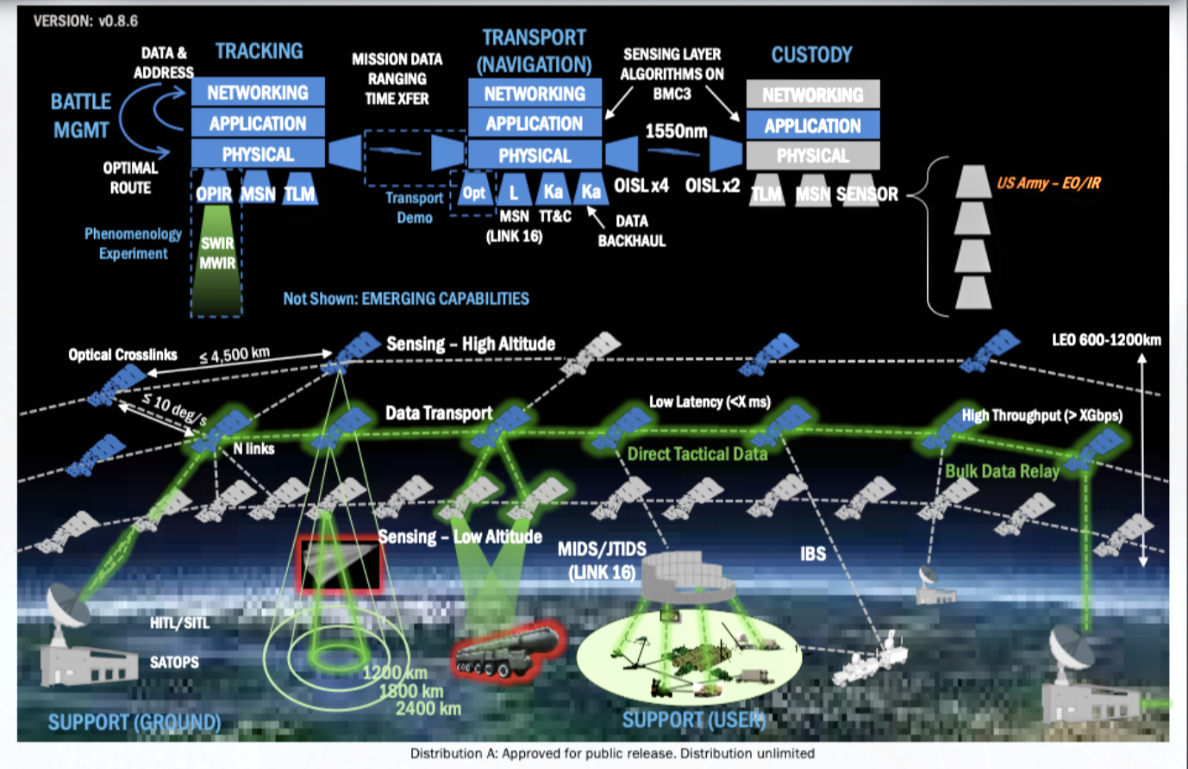
Missile warning mission uses a mix of space-based and terrestrial sensors. Satellite communications (SATCOM) systems facilitate beyond line of sight connectivity. Terrestrial and space environmental monitoring support to joint operations gives the joint force commander (JFC) awareness of the operational environment (OE). Functions common to joint CCDR operations at all levels of warfare consist of seven basic groups: command and control (C2), intelligence, fires, movement and maneuver, protection, sustainment, and information. SSA assists C2 by characterizing the space environment, including the ground and link segment. SSA provides insight into an adversary’s employment of space systems. [10]
A limited war strategy is to protect and defend US and Allied interests in space. Secondary objectives include (1) the ability to negate especially critical adversary space systems that place joint and coalition forces at extreme risk during terrestrial operations; (2) the ability to reconstitute or build resiliency into space architecture; and (3) to continue supporting the joint terrestrial force with war-winning, space-based enabling capabilities such as the Global Positioning System, missile warning, and satellite communications [11].
Space Development Agency (SDA) recognizes the need to establish an “intellectual pipeline” to access ideas from across the community to inform the future of the National Defense Space Architecture (NDSA). SDA specifically seeks novel architecture concepts, systems, technologies, and capabilities that:
1. Enable leap-ahead improvements for future tranches of currently planned NDSA capability layers
2. Enable new capability layers to address other emerging or evolving warfighters needs.
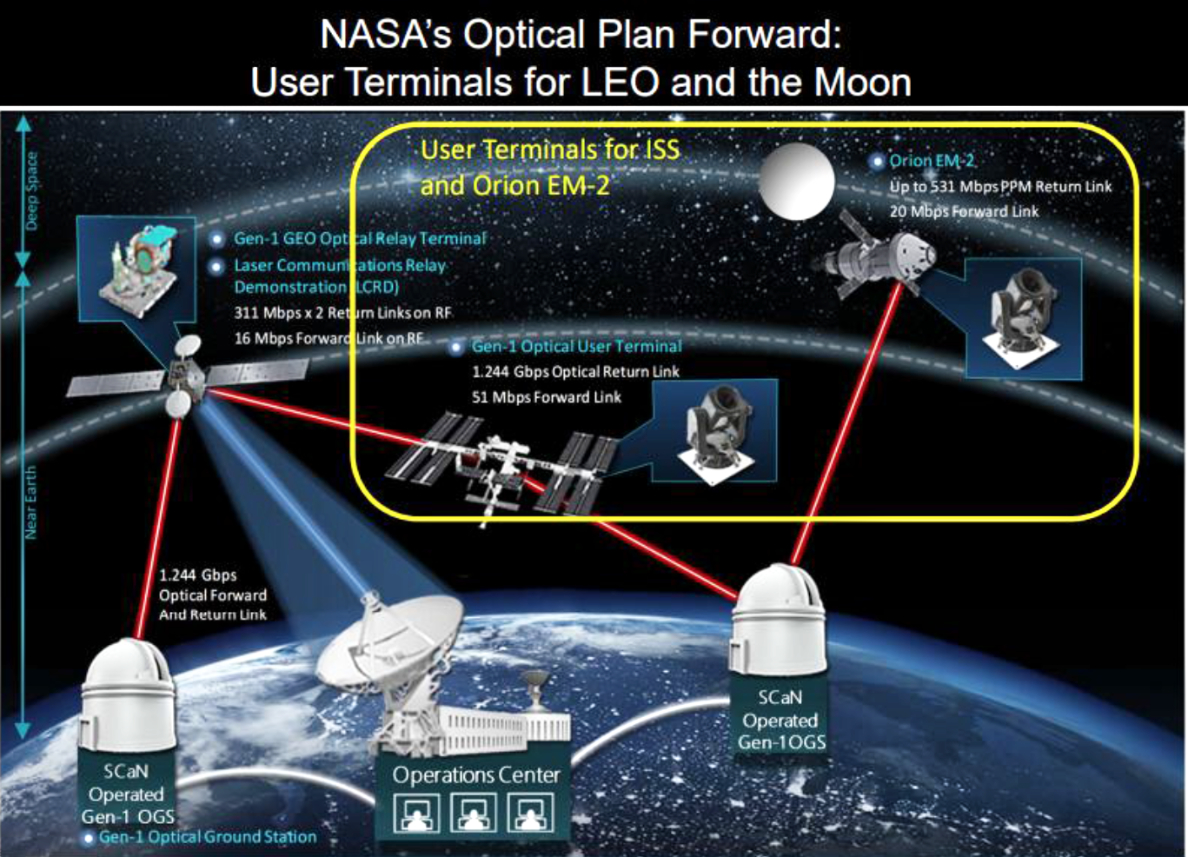
The year-old Space Development Agency (SDA) emphasizes speed in pursuing innovative space capabilities. [12] A proliferated Low Earth Orbit (LEO) constellation of satellites and sensors connect to the military’s tactical legacy datalinks and weapons systems to deter against advanced threats. In particular, beyond the line-of-sight targeting capabilities and enabling the detection, tracking and fire control of advanced missile threats would be a part of the system. The architecture is focused on delivering the initial capabilities of beyond the line-of-sight targeting for time-sensitive targets, both ground and maritime.
The SDA’s) effort to create and sustain resilient, affordable and lethal space-based capabilities as part of the NDSA for the military also provides the space-based backbone for the services’ Joint all-domain command and control, or JADC2. Specifically, the SDA is looking to field advancements across the transport, battle management, tracking, custody, deterrence, navigation and support layers of the NDSA. The agency also is addressing capability gaps in the military’s space solutions, including providing space situational awareness in extended space to cis-lunar space between the Earth and the moon’s orbit.
The backbone transport layer of hundreds of satellites in low-earth orbit (LEO) will all be optically connected to form a mesh network in space. SDA will fly about 20 transport satellites to form the network and enable communications to legacy tactical datalinks. The data transport layer will talk directly via existing tactical datalinks (e.g., Link 16), down to weapons systems that are already fielded and already have such capability (e.g., Army’s TITAN ground system).
Three sensing layers will feed data to the transport layer:
(1) a tracking layer made up of overhead persistent infrared (OPIR) sensors to detect and track adversarial advanced missiles
(2) a custody layer that would allow military users to send target location information in real time directly to weapons systems
(3) a deterrence layer for space situational awareness. The sensing satellites will detect the missile; send the track to the transport layer where that data could be fused with other data and then sent down to the ground to the actual weapon systems that would engage the threat.
The SDA would fly eight wide field-of-view sensors in Tranche Zero to demonstrate the traceability used for hypersonic glide vehicle detection over the entire globe, as well as for passing the data either directly to the ground or to the transport satellites.
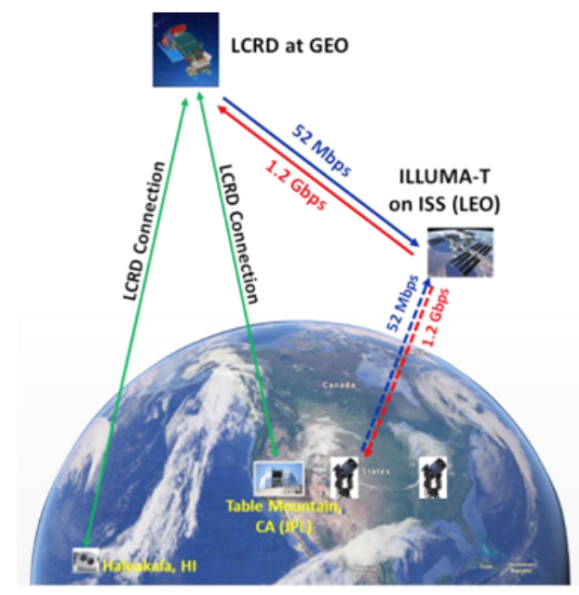
Figure 7. Illuma-T Operational Characteristics. Primary Mission
Requirements is to Communicate Through LCRD.
For the custody layer, the SDA will rely on mission partners—the Army, Air Force and other agencies—to build out ISR satellites to provide the beyond-line-of-sight targeting capability. In Tranche Zero, the SDA is working to show that it can move data to the ground from the mission partners, send data from the partners directly back up to the SDA transport satellites, and then send that data over the mesh network to other areas of interest.
“For example, if the Army had one of their TITAN ground stations and they have that located in Germany, they could download the data to that system in Germany, send it back up to transport, where then it would be used by transport satellites to go down to an F-35 in the INDOPACOM Theater via Link 16,” Derek Tournear, Director of the SDA clarifies. “That’s one example that we’re going to demonstrate in Tranche Zero.”
For the third sensing layer, the SDA is conducting studies on how to best design a deterrence solution that provides detection and tracking beyond geosynchronous orbit out to cis-lunar space. And that sensing would feed data back into the transport layer. Operating in that environment has historically been ignored due to a lower priority over items that are in LEO or geosynchronous.
A battle management layer of the NDSA architecture would tie all the layers together and would be where onboard processing, algorithms for autonomous operations and any automatic target recognition algorithms would run.
“In order to do those missions, essentially the autonomous detection and tracking, and targeting of time-sensitive targets and missiles, there is a lot of processing that needs to be done,” Tournear clarifies. “That processing will reside ultimately on board the sensing satellites as well as the transport satellites. That’s our battle management layer.”
Moreover, the position, navigation and timing (PNT) data of the NDSA transport layer offers an additional PNT signal to individual users that are tied into the associated communications network. To help enable the military’s JADC2 operations, the NDSA platform would connect to all of the services’ ground and air networks tied into JADC2. [13]
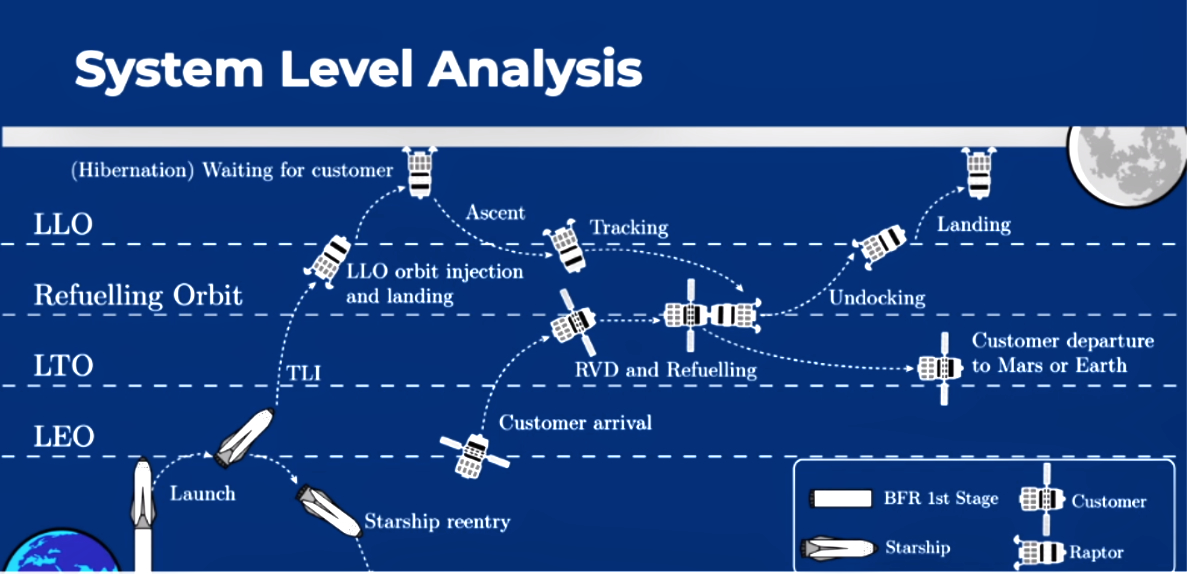
Figure 8. Click to enlarge.
The US military has come to rely on space-enabled capabilities ranging from communications to the Global Positioning System (GPS), in order to achieve battlefield effectiveness. Many systems and units cannot function without space-enabled capabilities, and this has created a vulnerability that near-peer adversaries of the US and its allies are unlikely to ignore. In other words, space is becoming “increasingly, congested, contested, and competitive.” [14]
The US is a space-dependent nation when it comes to military operations. Air Force Space Command (AFSPC) focuses on deterrence both in space and terrestrially and exercises both offensive and defensive capabilities. In negating adversary counter-space systems, all parties are likely augment space-based capabilities with local terrestrial equivalents like high-altitude long-endurance drones, pseudolites for position, navigation, and timing (PNT), terrestrial radios, fiber lines, or commercial intelligence, surveillance, and reconnaissance (ISR) capabilities. Additionally, these conceptual systems defend vital orbital regimes or points in space, such as Molynia orbits, certain sections of the geosynchronous belt, cis-lunar and lunar orbits, and earth-moon LaGrange points. LEO orbits are too numerous and inclinations too varied to actively defend with “cruisers,” except for perhaps some sun-synchronous orbits.
Within the rest of LEO, defenses on-board the high-value asset seems best suited to that orbital regime. Their cheap and in-depth maneuverability applies best in escorting high valued systems to new mission-dependent sites. [15] The development of agile, responsive next-generation space architecture will counter near-peer efforts to contest or deny US space-based systems. The notional architecture SDA developed is predicated on the availability of a ubiquitous data and communications transport layer and assumes the use of small, mass-produced satellites.
The architecture includes seven layers:
Space transport layer: A low-latency data and communications proliferated “mesh” network to provide 24/7 global communications.
Tracking layer: To provide early warnings of advanced missile threats
Custody layer: To keep watch over time-critical targets
Deterrence layer: To provide space situational awareness of, and access, to the cislunar space
Navigation layer: To create an alternative positioning, navigation and timing system for GPS-denied environments
Battle management layer: An artificial intelligence system to help deliver space sensor-derived data directly to tactical users
Support layer: Mass-producible ground command and control systems, user terminals and and rapid-response launch services
The Army has roughly 100 tactical ground stations, 13 operational ground stations and a few other dissemination vehicles to inform battlefield commanders. Tactical Intelligence Targeting Access Node (TITAN) aims to consolidate much of those capabilities to better provide "deep sensing" information from intelligence, surveillance and reconnaissance sensors from all domains.
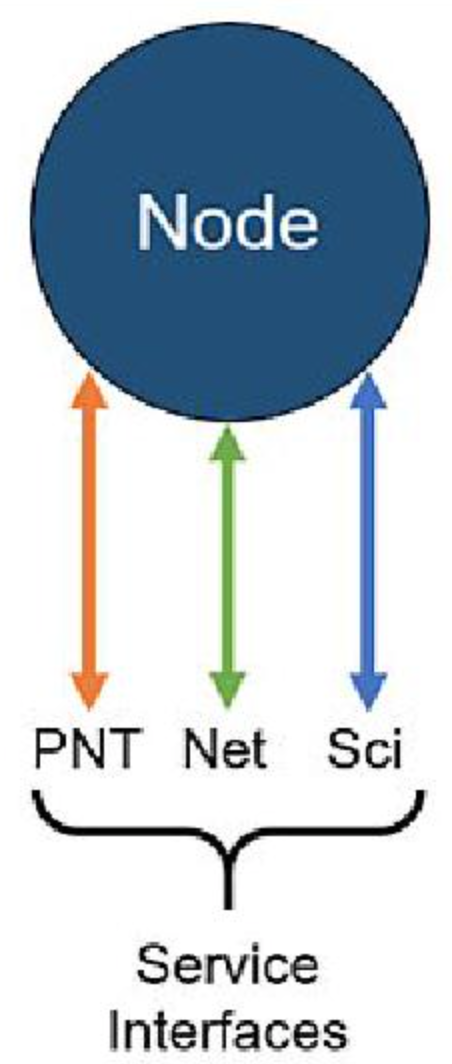
Figure 9. A Lunanet Node
with its Standard Service
Interfaces.
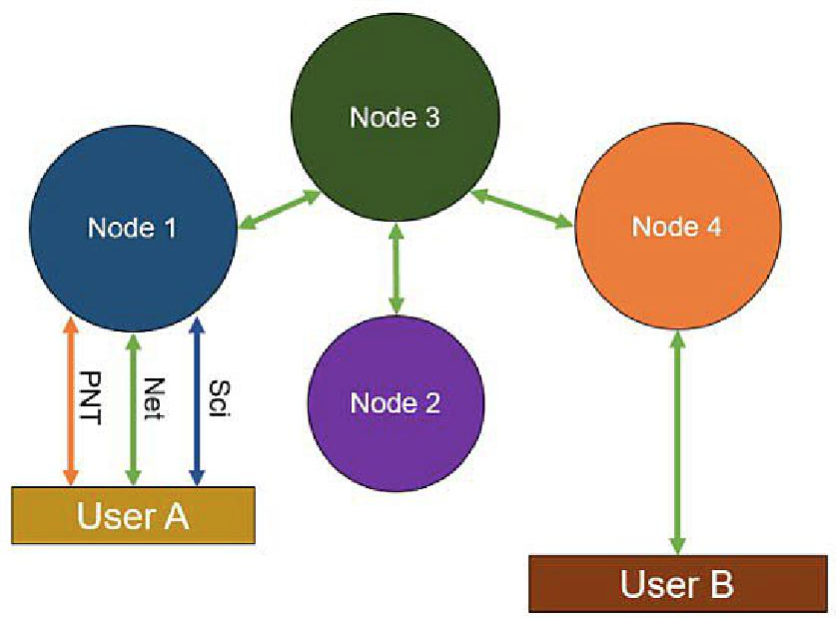
Figure 10. User A receives networking, PNT, and science services through
node 1 and is able to communicate with user B through Lunanet.
The scalable system is an expeditionary intelligence ground station, leveraging space and high altitude, aerial and terrestrial layer sensors to provide targetable data to fires networks. It also provides multi-discipline intelligence support to targeting, situational awareness, and understanding for mission command. Since the sensors will compile massive amounts of data, TITAN will need to rely on artificial intelligence and machine learning to sift through it. [16]
SDA head Fred Kennedy presented this notional satellite architecture at Space Symposium on April 9, 2019. He wants to launch the first satellites for the transport layer in 2022.
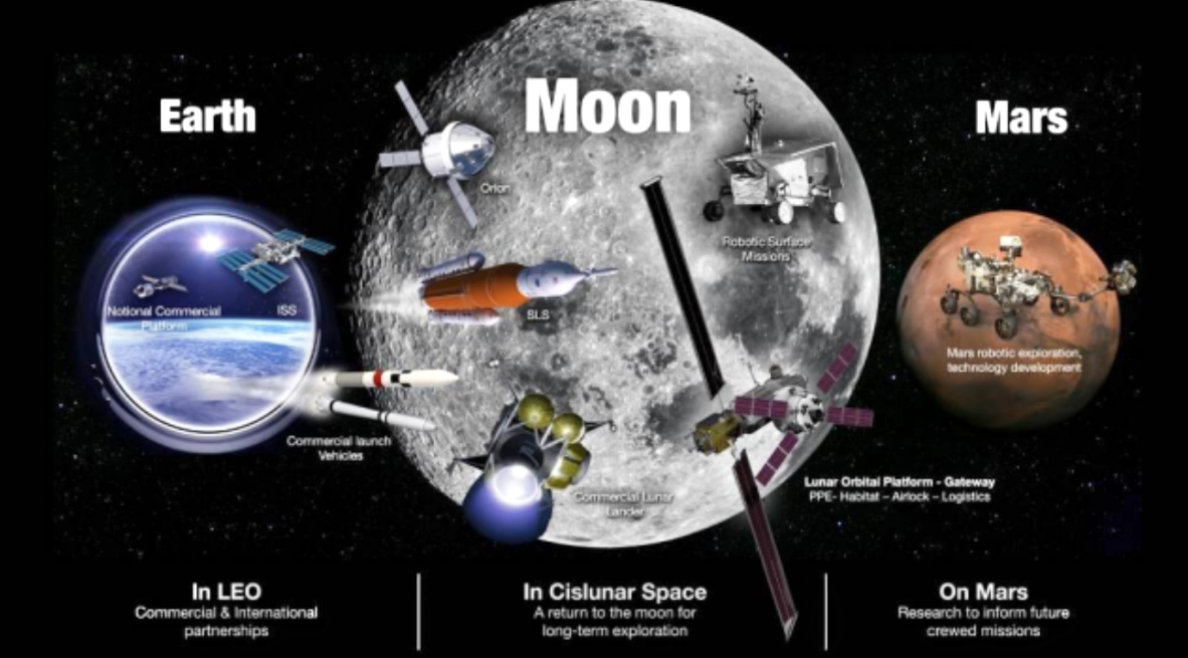
Figure 11. Click to enalrge.
SDA plans to roll out a “tracking layer” of about 200 satellites meant to provide global coverage of advanced missile threats using infrared imaging. Then, the SDA will launch a “custody layer” of about 200 satellites that use radar, electro-optical/infrared cameras and signals intelligence to sense and monitor objects on Earth—potential targets of interest, and how they may be moving or changing. The SDA’s notional architecture proposes a four part deterrence layer.
The first part, located in LEO, would be made up of outward facing space situational awareness sensors capable of providing data on deep space objects located immediately beyond geosynchronous orbit [17]. Additionally, development of a number of “advanced maneuvering vehicles” (i.e., X-37 successor or another spacecraft) promises to move between the Earth and the moon more quickly and efficiently than an adversary could, and deliver effects, potentially even weapons. [18]
Case Study
Set in the year 2026, this wargame, named in honor of retired General Bernard A. Schriever, explored critical space and cyberspace issues in depth and investigated the military utility of emerging space systems and cyberspace capabilities.
Explore and assess the resilience of a future architecture in a contested, degraded, and operationally limited environment
Identify processes; concepts of operations (CONOPS); and opportunities for tactics, techniques, and procedures (TTPs) development within a future architecture to improve defense and mutual support of all elements of National Security Spac
Examine how future anti-access and area denial (A2/AD) force structures will affect requirements for Air Force space operations and services.
This wargame highlighted the resilience of a future space architecture that incorporated characteristics of increased flexibility, maneuverability, and situational awareness, as well as the crucial role that U.S. allies and the commercial sector play in space and cyberspace capabilities. Key take-aways from the game include the importance of multi-domain awareness and integration, the warfighting value of our allies, and the operational contributions of commercial space.
As the wargame unfolded, a regional crisis quickly escalated, partly because of the interconnectedness of a multi-domain fight involving a capable adversary. The wargame participants emphasized the challenges in containing horizontal escalation once space control capabilities are employed to achieve limited national objectives. Approximately 175 military and civilian experts from government agencies around the U.S, as well as Australia, Canada, and the United Kingdom participated in the wargame [19].
GEO-Moon-Mars (Deep Space Exploration) Notional Satellite Architecture
GEO space-ground signals cross mostly cloud-free zones, wherein atmospheric turbulence causes sudden drops of signal lasting for many milliseconds. Unlike the growing space domain of GEO-Earth (Military) Notional Satellite Architecture with the extremely narrow divergence of the beams challenging Pointing, Acquisition, and Tracking (PAT) across the vast distances of space, Deep Space Network (DSN) has developed a new hybrid RF and optical antenna, capable of doing both RF and optical spacecraft passes with the same aperture [20]. Space Communications and Navigation (SCaN) serves as the Program Office for all of NASA’s space communications activities. SCaN manages and directs the ground-based facilities and services provided by the Deep Space Network (DSN), Near Earth Network (NEN) and Space Network (SN), and the SN's space segment, the Tracking and Data Relay Satellites (TDRS). NASA’s Plan of 2017 was for an Orion Exploration Mission-2 near the lunar orbit with data transfer directly to SCaN.
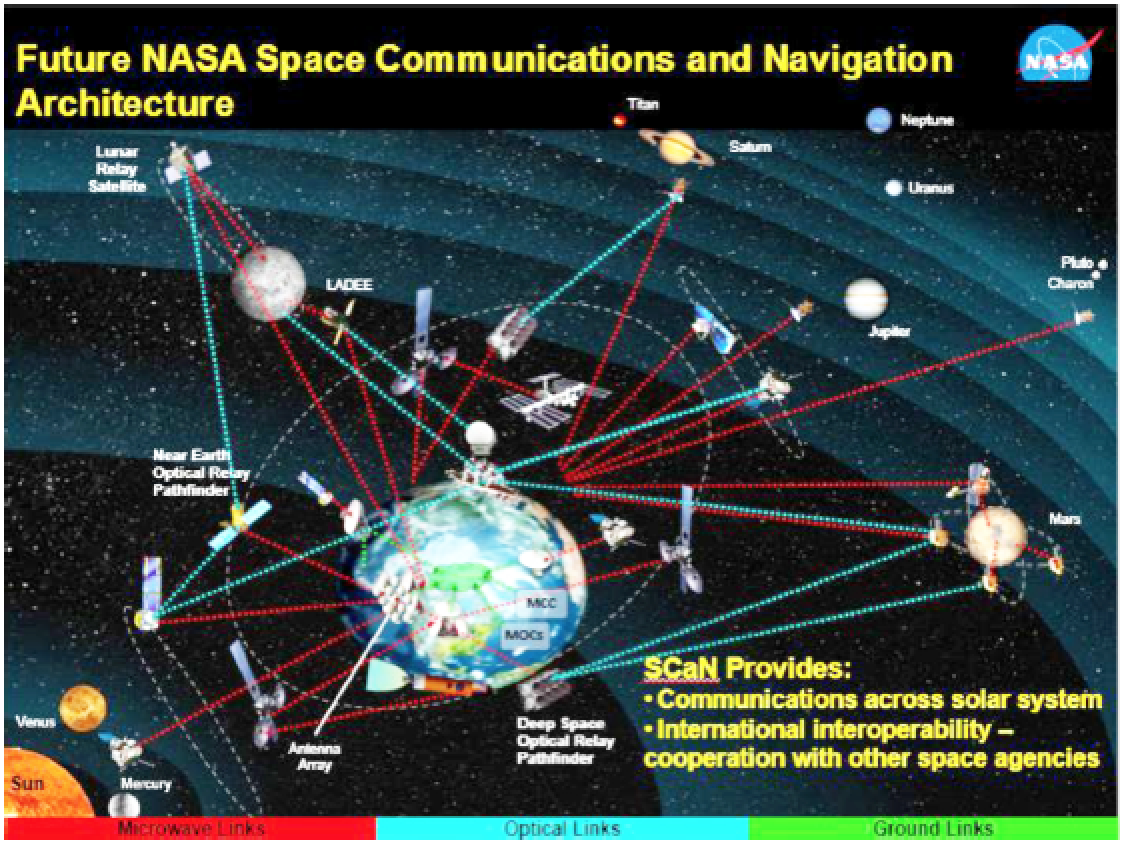
Figure 12. Click to enalrge.
The optical plan also included two user terminals for inter-satellite communications and data transfer to ground stations and ISS. However, data rates per deep space missions will increase by about one order of magnitude per decade for the next 50 years. The next two orders of magnitude are predicted to come from the introduction of deep space optical communications [21].
Improvement will result from Ka-band communication (about 100 Mb/s) for the LRO mission and laser communications (up to 622 Mbps) from Lunar Atmosphere and Dust Environment Explorer (LADEE) mission [22]. Commercial companies have a significant interest in free-space optical communication (FSOC) with world governments having invested in the initial infrastructure while retiring the technical risks on integrated photonics technology. Integrated photonics drove the costs of FSOC to well below those of RF systems in order for commercial networks of “fiber-in-the-sky” to realize fiber optic transmitter and receiver subsystem for the generation, coding, amplification and reception of light [23].
Designed to demonstrate high-bandwidth, bidirectional optical communications relay services between GEO and Earth, Laser Communications Relay Demonstration (LCRD, 2020) mission consists of two independent laser communication terminals, connected via a new electronic switch to provide high-speed frame switching and routing between the two optical space terminals (OSTs) while also serving as the interface to the host spacecraft.
The culmination of the LCRD mission will be a demonstration of a “space relay” communications link from a spacecraft LEO up through LCRD in GEO and then down to the ground. Specifically, NASA is
developing a new optical terminal to demonstrate on the international space station (ISS) in 2020 that is interoperable with LCRD for future space users in LEO or higher.
This next-generation terminal will leverage recent developments in integrated photonics, which should lower SWaP and cost of the flight modem by an order of magnitude relative to RF systems. The new terminal may even replace the current LCRD terminal design for GEO if radiation requirements can be met. [24] (See Figure 7.)
Designed to provide the significant capability required for human deep-space exploration, NASA’s Space Launch System (SLS) also provides a unique opportunity for lower cost deep-space science in the form of small-satellite secondary payloads. This opportunity will be leveraged beginning with the rocket’s first flight; a launch of the vehicle’s Block 1 configuration, capable of delivering at least 26 metric tons (t) to trans-lunar injection (TLI), which will see the Orion crew vehicle travel around the moon and return to Earth. On that flight, SLS will also deploy thirteen 6U CubeSat-class payloads to multiple destinations in deep space.
The EM-1 smallsat, one of four payload missions, is a Near Earth Asteroid Scout mission (MSFC) equipped with a solar sail to rendezvous with an asteroid and gather detailed imagery data.
Second, Lunar Flashlight mission (JPL) will look for ice deposits and identify locations of extractable resource lunar sites. BioSentinel mission (Ames Research Center), a yeast radiation biosensor, will measure effects of space radiation on DNA.
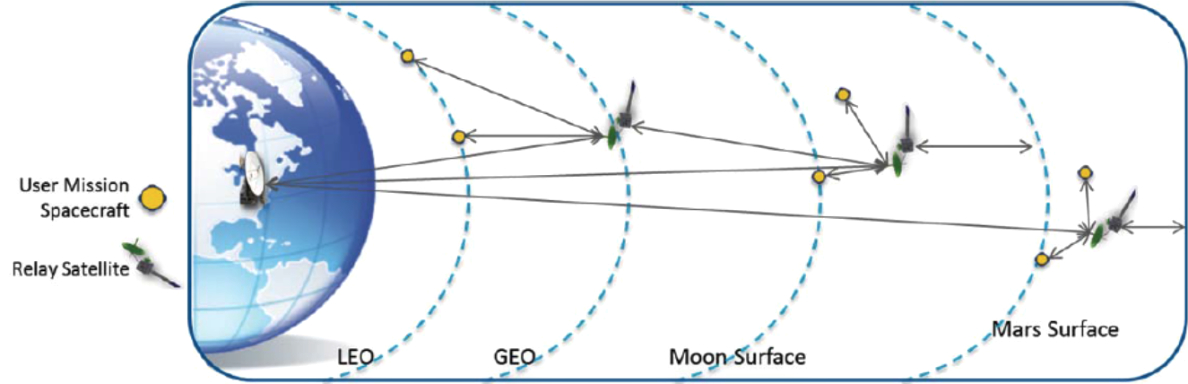
Figure 13. MAPS concept of operations: Space-based relay communication infrastructure concept. Click to enalrge.
And, Lunar IceCube mission (Morehead State University) will search for water in ice, liquid and vapor forms as well as other lunar volatiles, using a compact infrared spectrometer.
LunIR mission (Lockheed Martin) performed lunar flyby using a Mid-Wave Infrared (MWIR) sensor to collect spectroscopy and thermography data. From deep space beyond the moon, the CubeSat Mission to Study Solar Particles (CuSP) mission (Southwest Research Institute) will support space weather research by determining proton radiation levels during Solar Energetic Particle (SEP) events. [25]
Beyond GEO, some cislunar objects reside in low lunar orbit (LLO), a region within 100 km of the Moon’s surface. Since there is very little atmosphere on the Moon, objects can orbit at very low altitudes. The Moon has an uneven gravitational field. Consequently, satellites in LLO may have to make frequent station keeping adjustments to stay in orbit. The gravitational environment surrounding the Moon substantially limits operational capabilities for satellites and future space stations.
The Lagrange points are locations in space where the gravitational forces from two large bodies, like the Earth and the Moon, balance each other. Lagrange points are important because spacecraft or satellites can stay in a stable orbit without having to expend much fuel. The Earth-Moon Lagrange Points are strategically important because they are favorable locations to anchor commercial or military satellites and space stations. Or, the Lagrange points could serve as an appealing place to build trade and logistics terminals where crew or cargo arriving on spacecraft from Earth could be transferred to lunar landing vehicles. Alternatively, crew and cargo could launch from the lunar surface, dock with the station, and take a new vessel back to Earth.
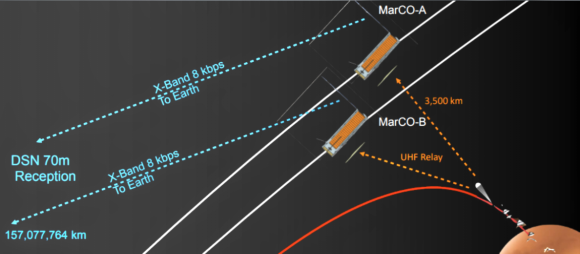
Figure 14. Click to enlarge.
Earth-Moon L 2 libration point satellites will provide navigation capability for the far side of the Moon. Grebow (2006) created architectures for continuous lunar south pole coverage by two satellites located in quasi-periodic orbits around the Earth-Moon L 1 and L 2. Romagnoli and Circi (2010) investigated Lissajous orbits around the Earth-Moon collinear libration points to realize a Lunar Global Positioning System (LGPS), The attractive L2, L4, and L5 locations for future positioning, navigation, and timing (PNT), SSA, and communications platforms will enable satellites to “see” behind the Moon, a part of cislunar space not visible from Earth.
The U.S. Space Force (USSF), in partnership with private vendors, plans development of a three-dimensional interface for lunaspatial intelligence (LUNINT) collection and a PNT constellation for cislunar space. [26] Lunar development serves as a critical proving ground for deeper exploration into the solar system [27]. NASA developed LunaNet architecture based on interconnected network nodes to create a terrestrial-like internet in space.
With the increase in human and robotic exploration at the Moon, space-based users, surface assets or lunar orbiters can use LunaNet’s network nodes as access points, analogous in functionality to terrestrial Wi-Fi routers and cellular towers. LunaNet architecture is based on nodes capable of providing a combination of the following three standard services, illustrated in Figure 9.
1. Networking Services (Net): Data transfer services capable of moving addressable and routable data units between nodes in a single link or over a multi-node, end-to-end path.
2. Position, Navigation, and Timing Services (PNT): Services for position and velocity determination, and time synchronization and dissemination. This includes search and rescue location services.
3. Science Utilization Services (Sci): Services providing situational alerts and science measurements for human and asset safety and protection. Science instrument data will also allow for further research, increasing return on investment overall.
LunaNet nodes may be connected together to provide the end-to-end path. In the example illustrated in the Figure 10 above, right.
User A, through Node 1 as its LunaNet access point, communicates with User B over multiple nodes providing networking services. Node 1 is simultaneously providing PNT and Science Utilization Services. The functions of an individual node within the larger architecture would influence amount of capabilities for each service type required for that node. The combination of nodes could be a heterogeneous set of assets:
1. Commercial, government, international, etc.
2. Spacecraft in any orbit or surface elements
3. Dedicated spacecraft or hosted payloads.
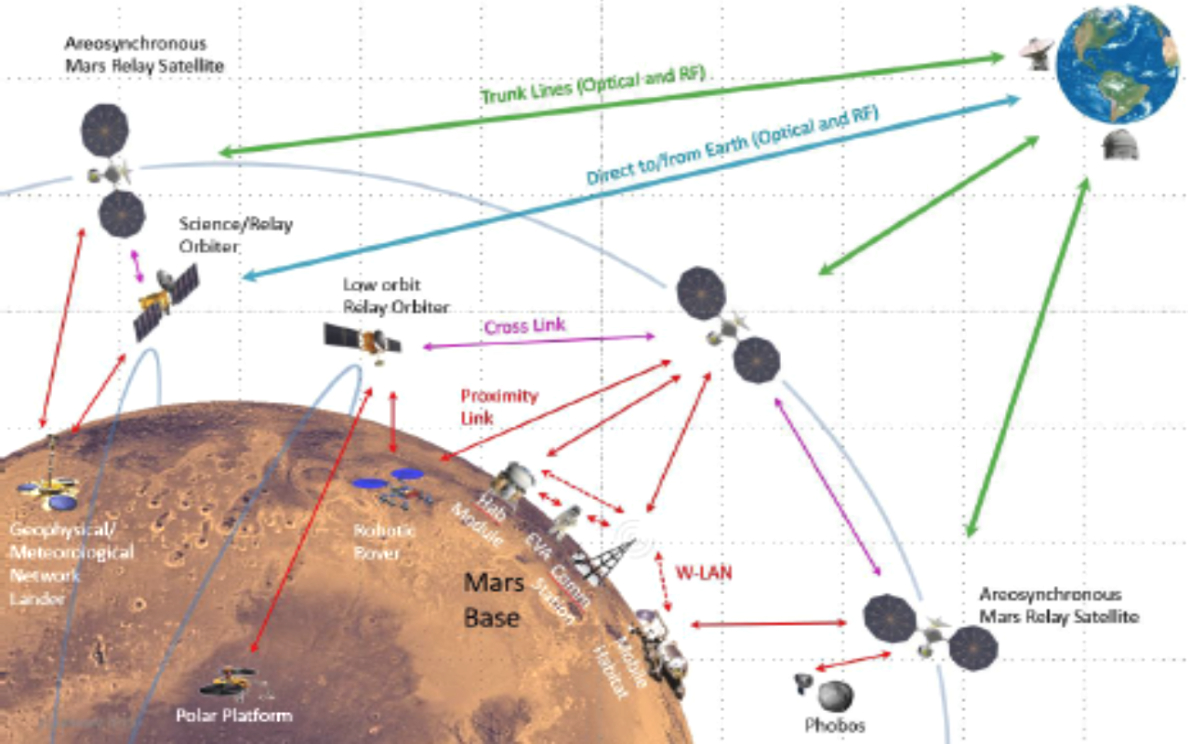
Figure 15. Notional Mars Architecture. Click to enlarge.
The architecture will aggregate links, funneling different sources of data to come together to one point to get combined into a single stream of data, as appropriate, to more efficiently use available links and to minimize the total number of links required [28].
NASA’s long-term plans for deep-space exploration focus on returning astronauts to cislunar space and constructing the Lunar Orbital Platform-Gateway in preparation for exploration of Mars; opportunities for small satellite payloads will likely be a part of these deep-space exploration plans.
NASA has selected SpaceX of Hawthorne, California, as the first U.S. commercial provider under the Gateway Logistics Services contract to deliver cargo, experiments and other supplies to the agency’s Gateway in lunar orbit.
NASA will have multiple supply missions in which the cargo spacecraft will stay at the Gateway for six to 12 months at a time. These firm-fixed price, indefinite delivery/indefinite quantity contracts for logistics services guarantee two missions per logistics services provider with a maximum total value of $7 billion across all contracts as additional missions are needed.
As defined by the Exploration Systems Architecture Study (ESAS), the lunar architecture is a combination of the lunar “mission mode,” the assignment of functionality to flight elements, and the definition of the activities to be performed on the lunar surface. The trade space for the lunar “mission mode,” or approach to performing the crewed lunar missions, was limited to the cislunar space and Earth-orbital staging locations, the lunar surface activities duration and location, and the lunar abort/return strategies.
The mission mode analysis was built around a matrix of lunar- and Earth-staging nodes. Lunar-staging locations initially considered included the Earth-Moon L1 libration point, Low Lunar Orbit (LLO), and the lunar surface. Earth-orbital staging locations considered included due-east Low Earth Orbits (LEOs), higher-inclination International Space Station (ISS) orbits, and raised apogee High Earth Orbits (HEOs). Cases that lack staging nodes (i.e., “direct” missions) in space and at Earth were also considered [29].
As defined by the Exploration Systems Architecture Study (ESAS), the lunar architecture is a combination of the lunar “mission mode,” the assignment of functionality to flight elements, and the definition of the activities to be performed on the lunar surface. The trade space for the lunar “mission mode,” or approach to performing the crewed lunar missions, was limited to the cislunar space and Earth-orbital staging locations, the lunar surface activities duration and location, and the lunar abort/return strategies. The mission mode analysis was built around a matrix of lunar- and Earth-staging nodes. Lunar-staging locations initially considered included the Earth-Moon L1 libration point, Low Lunar Orbit (LLO), and the lunar surface [30].
Case Study
Imagine you’re in a spacecraft heading from the Earth to the moon. At what point do you stop depending on the network of Earth- and space-based sensors that tell you what’s nearby, and start depending on a separate network that offers situational awareness near and on the moon? If you want to collect signals intelligence from systems emitting near the moon, what orbit do you need to be in, and how do you ensure the data can traverse a much farther distance than information in closer orbits? Those considerations are among the questions Space Force’s intelligence officers are asking.
Rhea Space Activity and Australia-based Saber Astronautics, co-founders of LUNINT, will create a three-dimensional dashboard that shows the coordinates of noteworthy objects in cislunar orbit, furthering the practice of space domain awareness.
Saber aims to introduce LUNINT into the Space Force’s virtual-reality “Space Cockpit” software that was developed by the 460th Space Wing at Buckley Air Force Base, Colo, as well as the commercial Predictive Ground Station Interface software.
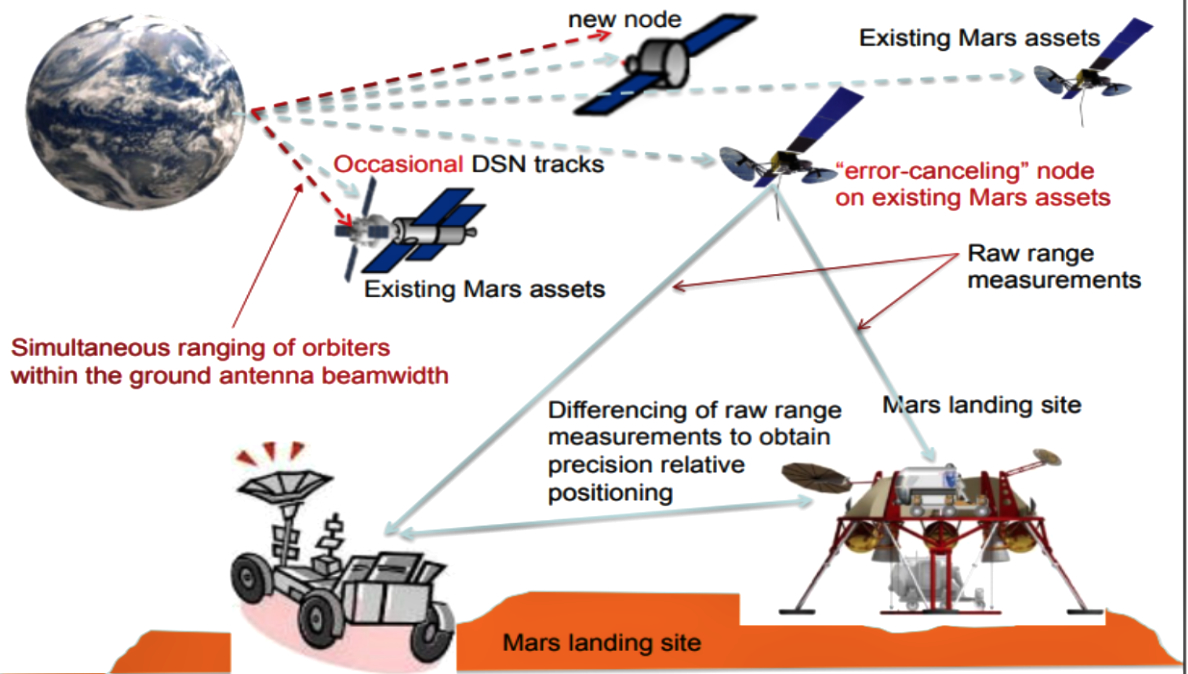
Figure 16. Schematic of MRNSS architecture: Key concept of in-situ regional navigation services — differencing of range measurements to eliminate common bias. Click to enalrge.
Tools such as virtual reality enable space professionals to envision battlespace within which their satellites operate. They can turn and look at where other satellites are relative to them and learn what the other satellite is doing, why it is so near, and how I should respond in face of such a real experience.
The intuitive feel for battlespace has previously been lacking on a text computer screen. Cislunar propagators are algorithms that help and predict a spacecraft’s orbital location and utilize decades of archived lunar images to map the moon’s surface. Additionally, the LUNINT program will indicate optimum satellite constellation architecture to monitor cislunar spacecraft and recommend how LUNINT capabilities will fit into current US intelligence practices.
As humans return to the moon, it may require its own sort of GPS enterprise, communications, remote sensing, and more that the Earth already uses. The USSF will want to track all of that activity as it expands, ideally on a daily basis to keep tabs on where satellites, debris, and other objects are going. Space users and travelers need to establish norms of behavior to keep the amount of space debris down in new orbits, so there’s less to worry about for space domain awareness. [31]
The increased dependency on ground-based navigation support for an Earth-directed domain, renders a need for Earth outward-looking domain toward deep space exploration. The current baseline system for deep space navigation utilizes Earth-based radiometric tracking, requiring long-duration observations to perform orbit determination and generate a state update.
The Multi-spacecraft Autonomous Positioning System (MAPS) takes advantage of the growing inters- spacecraft communication network and infrastructure to enable network-based space navigation. A notional concept of operations shows the use of the Mars Reconnaissance Orbiter and Mars Odyssey spacecraft as relays for surface assets. The growth of this communications architecture is continued through MAVEN, and future potential commercial Mars telecom orbiters. This growing network provides an initial Mars local capability for inter-spacecraft communication and navigation. [32]
Many space communications and exploration strategies relate to sending robotic and human missions to destinations beyond Low Earth Orbit (LEO), including cis-lunar space, Near-Earth Asteroids (NEAs), the Moon, and Mars and its moons. NASA’s Space Communications and Navigation (SCaN) program office provides communication and tracking services to space missions during launch, in-orbit testing, and operation phases. Currently, SCaN’s space networking relay satellites mainly provide services to users below GEO, at Near Earth Orbit (NEO), below LEO, and in deep space.
The potential exists for using a space-based relay satellite, located in the vicinity of various solar system destinations, to provide communication space links to missions both below and above its orbit. Such relays can meet the needs of human exploration missions for maximum connectivity to Earth locations and for reduced latency. Relay satellites in Earth through cis-Lunar orbits are normally located in stable orbits requiring low fuel consumption. Relay satellites for Mars orbit are normally selected based on the mission requirement and projected fuel consumption. Relay satellites have extreme commonalities of functions between them, differing only in the redundancy and frequencies used.
The mission design methodology developed by the Collaborative Modeling for Parametric Assessment of Space Systems (COMPASS) team13 was used to produce the satellite relay design and to perform various design trades [33]. The report includes a detailed design of the spacecraft, a description of each sub-system and its properties, a mission feasibility determination based on the design effort, a list of possible launch vehicles, the total mass and power requirement during the various phases, and a final cost estimate for the entire mission, including non-recurring engineering costs.
As human space exploration pushes back toward the moon and onward to Mars, the architectures for extended operations are continuing to mature and move towards implementation. As part of these missions, including human landers and ascent vehicles, an increased infrastructure will need to be deployed to support long duration scenarios. One aspect of this is the ability to communicate and navigate across the lunar surface. To enable this, a Lunar-wide communication network will be deployed, sizing up with the amount of bandwidth required for operations. This network can also be used for navigation as well. [34]
The Martian communication network, along with deep space network support, provides an initial architecture for simulation and analysis of MAPS, providing a notional deep space implementation. This scenario is used for initial trade studies to determine capability assessments and sensitivity analysis.
This architecture also serves as the mission scenario capturing the ideal initial deep space implementation of MAPS [35The fastest path to flight is to take advantage of the maturing cubesat community, with continuing expansions out into deep space, being demonstrated through the Secondary Payloads on the SLS Artemis I flight.
This approach has also already been successfully implemented and operated for Martian missions. Recently, NASA/JPL landed Insight on the surface of Mars. This mission included two companion spacecraft MarCO-A and MarCO-B, whose primary mission was two-fold: demonstrate the capability for cubesats to operate in deep space, and provide a communication relay for high-rate data collection during the lander’s descent operations. [36]
The MarCO (Mars Cube One) spacecraft launched with the InSight mission from Vandenberg AFB on May 5, 2018. These spacecraft, the first interplanetary CubeSats, serve as technology demonstrators, supporting the InSight Mars lander. During InSight’s entry, descent, and landing sequence, the MarCO spacecraft will flyby Mars, collecting transmitted data from the lander, and relaying it back to the Deep Space Network (DSN) on Earth.
This serves as a demonstrator for the “carry-your-own-relay” concept that might be utilized on more challenging future missions Prior to InSight support, the mission will also demonstrate the capability for a CubeSat sized, DSN compatible deep space transponder, to independently navigate from the Earth to Mars with a small spacecraft, and flight testing for numerous commercial products.
This marked the first time cubesats had been launched on an interplanetary voyage, the first time three vehicles would be flying to Mars in loose proximity, and the first time secondary spacecraft have been used as a “carry-your-own-relay” concept for critical operations (InSight EDL) [37]. A low-Earth orbit (LEO) demonstration mission concept was developed and analyzed for MAPS.
This mission scenario focused on capturing the in-flight accuracy of the spacecraft clocks as well as in-flight packet transmission, and state estimation among a limited number of assets. To support this mission, both software and hardware simulation tools were developed. The simulation architecture allowed for analysis of link budgets and estimated performance as a function of individual asset orbits and simulated errors (such as external perturbations and timing uncertainty). [38]
As the orbiting and surface elements build up in the vicinity of the Mars landing site, traditional methods that require pairing one dedicated ground station with one spacecraft to generate tracking measurements becomes impractical. This is due to a large number of flight assets in the same vicinity. Moreover, the Earth-based tracking approach is limited by the speed of light. As a spacecraft travels further from Earth, the communication lag time due to the finite speed of light increases.
Deep Space Network (DSN), a shared resource for many deep space spacecraft, operates navigation of all spacecraft to Mars using the large antennas from NASA ground stations on Earth. Therefore, the tracking time to perform precision orbit determination (OD) for four or more Mars orbiting navigation satellites would be challenging, if not impossible.
Human Mars explorations require substantial buildup of orbiting and surface infrastructures on Mars. In addition to communication coverage, location awareness is essential to supporting various human and robotic activities on the Mars surface and on orbit. This could include localizing discoveries and returning to sites, construction/assembly of structures and habitats, entry/descent/landing, approach/rendezvous/docking, Mars ascent, and orbit insertion, and so on.
A favorable Mars relay network architecture option is to have two aerostationary orbiters that are within the line-of-sight of the landing site, and yet have enough angular distance such that at least one of the orbiters would be able to provide continuous communication coverage to the landing site during solar conjunction events. A deep space habitat (DSH) has been proposed, which would orbit around Mars in an inclined 48-hour circular orbit. The DSH would act as a staging and monitoring facility for the human Mars surface explorations, and to perform tele-robotic activities on Mars, Phobos, and Demos [39]. (See Figure 15.)
Taking into account the need to provide only regional coverage on the Mars surface, and the expected deployment of areostationary relay orbiters and other orbiting assets that hover over the Mars landing site, we propose to leverage on the Mars orbiting infrastructure build-up to establish a Mars Regional Navigation Satellite System (MRNSS). With the augmentation of one or more low-cost dedicated Mars navigation satellites that trace around a figure-8 path in areosynchronous orbits, there can be four or more navigation nodes with sufficient geometric diversity that could enable accurate localization in the vicinity of the Mars landing site.
Note a three or four ground stations “looking upward,” and tracking a constellation of spacecraft that is composed of one reference spacecraft and multiple target spacecraft operating at the geosynchronous orbit (GEO).
www.aiaa.org/
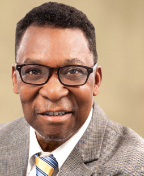
Ronald Freeman is the Vice-Chair of Space Operations and Support Technical Committee, AIAA (American Institute of Aeronautic and Astronautics). He is an investigative writer in the aerospace industry with more than ten years as a frequent presenter of technical papers at AIAA conferences. Ron completed his PhD in Technology Management at Northcentral University, MAS in Aerospace Science from Embry-Riddle Aeronautical University, and BA degree in Mathematics from the Defiance College (OH). Ron is an active member in the District of Columbia Science Writers Association and Rockville Science Cafe (MD).
NOTES AND REFERENCES
This section contains references as well as attributions for material quoted or edited for this report. For clarity, especially in the attributions, and to allow separated chapters to retain their references, some documents are referred to more than once.
[1]. Weigel, A. (2004). Space System Architecture Lecture 1: Space Systems and Definitions Framing Document. https://ocw.mit.edu/courses/ 2004/lecture-notes
[2] Hastings, D. & McManus, H. (2004). Space System Architecture: Final Report of SSPARC: The Space Systems, Policy, and Architecture Research Consortium (Thrust I and II).
[3] Townsend, B. A small revolution in space: An analysis of the challenges to US military adoption of small satellite constellations. https://apps.dtic.mil/dtic/
[4] Building a plan for NOAA’s 21st century satellite observing system (May 31, 2018)
[5] Davis, J., Mayberry, J., & Penn, J. (2019). On-orbit servicing: Inspection, repair, refuel, upgrade, and assembly of satellites in space.
[6] Singh, L., Whittecar, W., DiPrinzio, M., Herman, J., Ferringer, M., & Reed, P. (2020). Low cost satellite constellations for nearly continuous global coverage. Nature Communications, 11(200)
[7] Barnhart, D. & Rughani, R. (2020). On-orbit servicing ontology applied to recommended standards for satellites in Earth orbit. Journal of Space Safety Engineering, 7, 83–98
[8] Barnhart, D. & Rughani, R. (2020). On-orbit servicing ontology applied to recommended standards for satellites in Earth orbit.
[9] Lal, B., Blanco, E., Behrens, J., Corbin, B., Green, E., Picard, A., & Balakrishnan, A.. (2017). Global trends in small satellites. IDA Science & Technology, Policy Institute, Washington, DC.
[10] Scaparrotti, C. (2013). Joint Publication 3-14, Space Operations. US Department of Defense, Joint Chiefs of Staff, Washington, DC.
[11] Davenport, B. (2020). On implementing a space war-fighting construct. Air & Space Power Journal, 34(1), 63-74.
[12] Announcement seeks to develop “Intellectual Pipeline” for National Defense Space Architecture Original Response Date: Jan. 20, 2021
[13] Underwood, K. (August, 2020). Military aims to urgently provide disruptive satellite capabilities. Signal Magazine
[14] Townsend, B. (2011). A small revolution in space: An analysis of the challenges to US military adoption of small satellite constellations. National Security Space Strategy, Unclassified Summary, January 2011.
[15] Davenport, B. (2020). On implementing a space war-fighting construct. Air & Space Power Journal, 34(1), 63-74.
[16] Kimmons, S. (October, 2019). TITAN system being developed to tie 'deep sensing' to long-range fires Army News.
[17] Erwin, S. (July 4, 2019). Space Development Agency releases its first solicitation. Space News
[18] Singh, L., Whittecar, W., DiPrinzio, M., Herman, J., Ferringer, M., & Reed, P. (2020). Low cost satellite constellations for nearly continuous global coverage. Nature Communications 11, 200.
[19] Schriever Wargame Concludes (February 18, 2015). Air Force Space Command Public Affairs.
[20] Lichten, S.,Hoppe, D., Mohageg,M., Piazzolla,S., Amy Smith, A., & Soldan, H. (2019). Operational Concepts and Challenges for Optical communications in NASA’s Deep Space Network, In SpaceOps Workshop 2019, Montreal, Quebec.
[21] Cornwell, D. (2016). Space-based laser communications break threshold. Optics and Photonics News, 27(5), 24- 31.
[22] Stubbs, T., Glenar, D., Wang, Y., Hermalyn, B., Saranto, M., Colaprete, A., & Elphic, R. (2014). The impact of meteoroid streams on the lunar atmosphere and dust environment during LADEE mission. In 45th Lunar and Planetary Science Conference; March 17, 2014 - March 21, 2014; The Woodlands, TX.
[23] Robson, K. (2016). NYO experimental satellites. National Reconnaisssance Office, Chantilly, VA
[24] Schoolcraft, J., & Wilson, K. (2011). Experimental characterization of space optical communications with disruption-tolerant network protocols. In 2011 International Conference on Space Optical Systems and Applications (ICSOS) (pp. 248-252). IEEE
[25] Robinson, K. & McLemore, C., NASA’s Space Launch System: Deep-space opportunities for smallsats Document ID 20180005267
[26] Kaplan, S. (2020). Eyes on the Prize. The Strategic Implications of Cislunar S